Application of microfluidic chips in anticancer drug screening
DOI:
https://doi.org/10.17305/bjbms.2021.6484Keywords:
Microfluidic chip, anticancer drug screening, high throughput screeningAbstract
With the continuous development of drug screening technology, new screening methodologies and technologies are constantly emerging, driving drug screening into rapid, efficient and high-throughput development. Microfluidics is a rising star in the development of innovative approaches in drug discovery. In this article, we summarize the recent years' progress of microfluidic chip technology in drug screening, including the developmental history, structural design, and applications in different aspects of microfluidic chips on drug screening. Herein, the existing microfluidic chip screening platforms are summarized from four aspects: chip structure design, sample injection and drive system, cell culture technology on a chip, and efficient remote detection technology. Furthermore, this review discusses the application and developmental prospects of using microfluidic chips in drug screening, particularly in screening natural product anticancer drugs based on chemical properties, pharmacological effects, and drug cytotoxicity.
Citations
Downloads
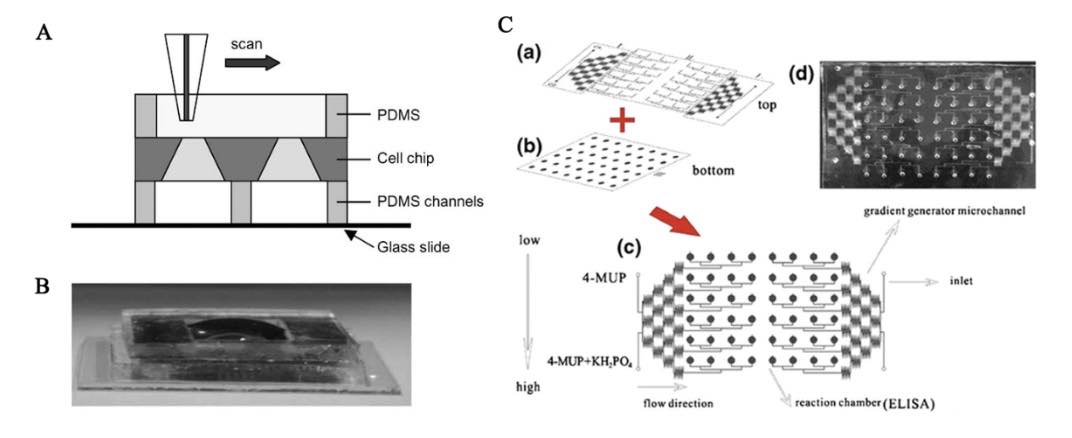
Downloads
Additional Files
Published
Issue
Section
Categories
License
Copyright (c) 2021 Xin-yue Fan, Zhuo-fen Deng, Yan-yan Yan, Valerii E. Orel, Andrii Shypko, Valerii B. Orel, Donika Ivanova, Christian Pilarsky, Jing Tang, Zhe-Sheng Chen, Jian-ye Zhang

This work is licensed under a Creative Commons Attribution 4.0 International License.
How to Cite
Accepted 2021-09-28
Published 2022-06-01